Bond Order In Molecular Orbital Theory: A Guide to Understanding
Bond order, a measure of the strength of a chemical bond, is a crucial concept for comprehending molecular structures. By utilizing Molecular Orbital (MO) theory, scientists can determine bond order, providing insights into the behaviour and properties of molecules. This guide explores the steps involved in calculating bond order using MO theory, elucidating its significance, historical context, and practical applications.
This article delves into the nuances of bond order determination, empowering researchers and students with a robust understanding of molecular bonding.
How to Find Bond Order Using Molecular Orbital Theory
Determining bond order using Molecular Orbital (MO) theory is pivotal for understanding chemical bonding and molecular structures. Key aspects of this process include:
- Molecular orbital construction
- Electron configuration
- Bonding and antibonding orbitals
- Electron density distribution
- Molecular energy levels
- Bond order calculations
- MO theory applications
- Limitations of MO theory
- Historical developments
These aspects encompass the theoretical foundations, practical applications, and historical evolution of bond order determination using MO theory. Understanding these aspects enables researchers to accurately predict bond strengths, molecular geometries, and chemical reactivity.
Molecular Orbital Construction
Molecular orbital construction is a fundamental aspect of determining bond order using Molecular Orbital (MO) theory. It involves combining atomic orbitals to form molecular orbitals that describe the electron distribution within a molecule.
- Linear Combination of Atomic Orbitals (LCAO): LCAO is a method for constructing molecular orbitals by combining atomic orbitals. The coefficients of the atomic orbitals in the LCAO determine the shape and energy of the molecular orbital.
- Symmetry: The symmetry of the atomic orbitals involved in the LCAO determines the symmetry of the molecular orbitals. Molecular orbitals with the same symmetry can interact with each other to form bonding or antibonding orbitals.
- Overlap: The overlap between atomic orbitals determines the extent of interaction between them. Greater overlap leads to stronger interactions and lower energy molecular orbitals.
- Energy Levels: The energy levels of molecular orbitals are determined by the energy levels of the atomic orbitals that form them. Bonding orbitals are lower in energy than the atomic orbitals from which they are formed, while antibonding orbitals are higher in energy.
Understanding molecular orbital construction is essential for accurately predicting bond order and understanding the electronic structure of molecules. It provides a framework for analyzing and interpreting chemical bonding and molecular properties.
Electron Configuration
Electron configuration plays a pivotal role in determining bond order using molecular orbital theory. It describes the distribution of electrons in atomic and molecular orbitals, providing insights into the electronic structure and chemical properties of molecules.
- Atomic Orbitals: Electrons occupy specific atomic orbitals within atoms, each with its unique energy level and shape. The number and type of atomic orbitals involved in bonding determine the molecular orbitals that are formed.
- aufbau Principle: Electrons fill atomic orbitals in order of increasing energy, starting with the lowest energy orbitals. This principle guides the construction of molecular orbitals and determines the electron configuration of molecules.
- Hund’s Rule: When multiple electrons occupy the same energy level, they tend to occupy different orbitals with parallel spins. This rule influences the spin multiplicity of molecular orbitals and affects the overall bond order.
- Pauli Exclusion Principle: No two electrons in an atom or molecule can have the same set of quantum numbers. This principle restricts the number of electrons that can occupy each molecular orbital and affects the bonding interactions between atoms.
Understanding electron configuration is crucial for accurately predicting bond order and comprehending the electronic structure of molecules. It provides a foundation for analyzing and interpreting chemical bonding and molecular properties.
Bonding and Antibonding Orbitals
In the context of molecular orbital theory, bonding and antibonding orbitals play a critical role in determining bond order. Bonding orbitals are formed when atomic orbitals overlap in-phase, leading to constructive interference and a decrease in energy. This results in a strengthened bond between the atoms involved. Conversely, antibonding orbitals are formed when atomic orbitals overlap out-of-phase, causing destructive interference and an increase in energy, which weakens the bond or leads to an antibonding interaction.
The number of bonding and antibonding orbitals formed depends on the symmetry and overlap of the atomic orbitals involved. Bonding orbitals are typically lower in energy than the atomic orbitals from which they are formed, while antibonding orbitals are higher in energy. The difference in energy between bonding and antibonding orbitals is crucial for determining the stability and properties of molecules.
Understanding bonding and antibonding orbitals is essential for comprehending chemical bonding and molecular structures. By analyzing the molecular orbitals of a molecule, chemists can predict bond order, bond strength, and other important molecular properties. Practical applications of this understanding include the design and synthesis of new materials, the development of drugs and pharmaceuticals, and the investigation of chemical reactions and processes.
Electron density distribution
Electron density distribution plays a pivotal role in determining bond order using molecular orbital theory. The electron density distribution within a molecule provides insights into the bonding interactions between atoms and the overall electronic structure. By analyzing the electron density distribution, chemists can predict bond order, which is a measure of the strength of a chemical bond.
The electron density distribution is directly related to the molecular orbitals of a molecule. Bonding orbitals have a higher electron density between the bonded atoms, indicating a stronger bond, while antibonding orbitals have a lower electron density, indicating a weaker bond or antibonding interaction. The bond order is directly proportional to the difference in electron density between bonding and antibonding orbitals.
Real-life examples of electron density distribution within the context of bond order determination include the analysis of diatomic molecules such as H2 and O2. By studying the electron density distribution of these molecules, chemists have gained a deeper understanding of the bonding interactions and the nature of the chemical bond.
The practical applications of understanding electron density distribution are vast. In materials science, electron density distribution analysis helps in the design and development of new materials with desired properties. In drug discovery, it aids in understanding the interactions between drugs and biological molecules, facilitating the development of more effective and targeted therapies. Furthermore, electron density distribution studies contribute to our understanding of chemical reactions and processes, providing insights into reaction mechanisms and the behavior of molecules under different conditions.
Molecular Energy Levels
Molecular energy levels play a crucial role in determining bond order using molecular orbital theory. They represent the quantized energy states that electrons can occupy within a molecule, providing insights into the stability, bonding, and reactivity of chemical species.
- Orbital Energies: The energy of molecular orbitals is determined by the interactions between atomic orbitals and the number of electrons occupying them. Bonding orbitals have lower energies than the atomic orbitals from which they are formed, while antibonding orbitals have higher energies.
- Energy Gaps: The energy difference between bonding and antibonding orbitals is known as the energy gap. A larger energy gap indicates a stronger bond and greater stability.
- Molecular Terms: The arrangement of electrons in molecular orbitals gives rise to molecular terms, which describe the overall energy state and multiplicity of the molecule. These terms are essential for understanding the spectroscopic properties and chemical reactivity of molecules.
- Excited States: When molecules absorb energy, electrons can be promoted to higher energy orbitals, resulting in excited states. The energy levels and transitions between excited states provide valuable information about the electronic structure and dynamics of molecules.
Understanding molecular energy levels is fundamental to accurately predicting bond order and comprehending the electronic structure of molecules. It provides a framework for analyzing chemical bonding, interpreting spectroscopic data, and predicting the reactivity and behavior of molecules in various chemical processes.
Bond Order Calculations
Bond order calculations are a pivotal aspect of determining bond order using molecular orbital theory. They provide quantitative measures of bond strength and stability, facilitating a deeper understanding of chemical bonding and molecular structures.
- Orbital Overlap: The extent of overlap between atomic orbitals directly influences bond order. Greater overlap leads to stronger bonds and higher bond orders.
- Electron Configuration: The number and arrangement of electrons in molecular orbitals affect bond order. The presence of unpaired electrons in antibonding orbitals can reduce bond order.
- Resonance: In resonance structures, electrons are delocalized over multiple bonds, resulting in fractional bond orders. Bond order calculations help determine the relative contributions of resonance structures.
- Molecular Properties: Bond order correlates with various molecular properties, including bond length, bond strength, and reactivity. Accurate bond order calculations aid in predicting and understanding these properties.
Overall, bond order calculations provide valuable insights into the nature of chemical bonds, enabling chemists to predict molecular structures, explain chemical reactivity, and design new materials with desired properties.
MO theory applications
Molecular orbital (MO) theory applications play a pivotal role in determining bond order, providing valuable insights into chemical bonding and molecular structures. These applications extend beyond theoretical calculations and encompass a wide range of practical implications.
- Materials Design: MO theory aids in the design of novel materials with tailored properties by predicting bond order and understanding the electronic structure of materials.
- Chemical Reactivity: Understanding bond order through MO theory helps predict the reactivity of molecules, facilitating the development of more efficient catalysts and drugs.
- Spectroscopy: MO theory provides a framework for interpreting molecular spectra, enabling the identification and characterization of molecules based on their electronic transitions.
- Quantum Chemistry: MO theory serves as a foundation for advanced quantum chemical methods, allowing for accurate calculations of molecular properties and the study of complex chemical systems.
In summary, MO theory applications encompass diverse areas, from materials science and drug design to spectroscopy and quantum chemistry. By harnessing the power of MO theory to determine bond order, scientists gain a deeper understanding of chemical bonding and molecular properties, unlocking new avenues for innovation and discovery.
Limitations of MO theory
Molecular orbital (MO) theory, while providing a powerful framework for understanding bond order, has inherent limitations that need to be considered when determining bond order using this theory. One key limitation is the assumption of independent electrons, which neglects electron correlation effects. This assumption can lead to inaccuracies in predicting bond orders, particularly in systems with strong electron correlation, such as transition metal complexes and molecules with unpaired electrons.
Another limitation of MO theory lies in its inability to accurately describe all types of chemical bonding. For example, MO theory struggles to explain the bonding in highly ionic compounds, where electrostatic interactions play a dominant role. Additionally, MO theory often fails to capture the subtleties of weak interactions, such as van der Waals forces and hydrogen bonding.
Despite these limitations, MO theory remains a valuable tool for determining bond order and understanding chemical bonding. By acknowledging and addressing its limitations, chemists can utilize MO theory effectively to gain insights into molecular structures and properties. In practice, MO theory is often combined with other theoretical approaches, such as density functional theory (DFT), to overcome these limitations and provide more accurate predictions of bond order and molecular behavior.
Historical developments
Historical developments in the understanding of bond order using molecular orbital theory have significantly shaped our current knowledge and approaches to this fundamental chemical concept. These developments encompass various aspects, including the evolution of theoretical frameworks, instrumental advancements, and the contributions of key scientists.
- Early Quantum Theories:
The foundation of molecular orbital theory can be traced back to the early quantum theories of Schrdinger, Heisenberg, and Dirac, which laid the groundwork for describing the electronic structure of atoms and molecules. - LCAO Approximation:
The linear combination of atomic orbitals (LCAO) approximation, proposed by John C. Slater and Linus Pauling, provided a practical method for constructing molecular orbitals from atomic orbitals, enabling the calculation of bond orders. - Hckel Theory:
Developed by Erich Hckel, Hckel theory simplified molecular orbital calculations for conjugated systems, providing insights into the electronic structure and bonding of aromatic compounds. - Density Functional Theory (DFT):
DFT, a more sophisticated theoretical framework, has emerged as a powerful tool for calculating bond orders and understanding chemical bonding, complementing traditional molecular orbital theory.
These historical developments have not only advanced our theoretical understanding of bond order but also influenced the development of experimental techniques, such as spectroscopy and crystallography, which provide experimental evidence for the electronic structure and bonding in molecules. The interplay between theoretical and experimental advancements has been crucial in the progress of this field, leading to a deeper comprehension of chemical bonding and molecular properties.
Frequently Asked Questions on Finding Bond Order Using Molecular Orbital Theory
This section addresses common queries and clarifies key aspects of determining bond order using molecular orbital theory.
Question 1: What is molecular orbital theory and how does it relate to bond order?
Answer: Molecular orbital theory describes the electronic structure of molecules by combining atomic orbitals to form molecular orbitals. Bond order, a measure of bond strength, can be determined by analyzing the molecular orbitals and their electron configuration.
Question 2: How do you construct molecular orbitals using the LCAO method?
Answer: The linear combination of atomic orbitals (LCAO) method involves combining atomic orbitals with appropriate coefficients to form molecular orbitals. The coefficients are determined by the overlap and energy of the atomic orbitals.
Question 3: What is the significance of bonding and antibonding orbitals in bond order determination?
Answer: Bonding orbitals result from constructive interference of atomic orbitals, leading to lower energy and stronger bonds. Antibonding orbitals arise from destructive interference, resulting in higher energy and weaker bonds or antibonding interactions.
Question 4: How does electron density distribution affect bond order?
Answer: The electron density distribution within a molecule provides insights into bond order. Higher electron density between bonded atoms indicates stronger bonds, while lower electron density suggests weaker bonds or antibonding interactions.
Question 5: What are the limitations of molecular orbital theory in bond order determination?
Answer: Molecular orbital theory assumes independent electrons and may not accurately describe all types of chemical bonding, such as ionic bonds or weak interactions like van der Waals forces.
Question 6: How can molecular orbital theory be applied in practical scenarios?
Answer: Molecular orbital theory finds applications in materials design, understanding chemical reactivity, interpreting spectroscopy data, and developing more accurate quantum chemical methods.
These FAQs provide a deeper understanding of the concepts and applications of molecular orbital theory in bond order determination. The next section will explore advanced topics and recent developments in this field.
Tips for Determining Bond Order Using Molecular Orbital Theory
This section provides practical tips to enhance your understanding and accuracy when determining bond order using molecular orbital theory.
Tip 1: Master the LCAO method. Proficiency in the linear combination of atomic orbitals (LCAO) method is crucial for constructing molecular orbitals.
Tip 2: Analyze molecular orbital energy levels. The energy difference between bonding and antibonding orbitals provides insights into bond order.
Tip 3: Consider electron density distribution. The electron density between bonded atoms indicates bond strength and can be visualized using contour plots.
Tip 4: Utilize MO theory software. Computational chemistry software can assist with complex molecular orbital calculations and provide visual representations.
Tip 5: Understand the limitations of MO theory. Be aware of the assumptions and limitations of MO theory, such as the neglect of electron correlation.
Tip 6: Combine MO theory with experimental data. Experimental techniques, such as spectroscopy, can validate and complement MO theory predictions.
Tip 7: Explore advanced MO theory methods. Density functional theory (DFT) and other advanced methods can provide more accurate bond order calculations.
Tip 8: Practice regularly. Regular practice and application of MO theory will improve your proficiency and deepen your understanding.
By following these tips, you can effectively determine bond order using molecular orbital theory, gaining valuable insights into chemical bonding and molecular structures.
The next section will explore applications of MO theory in various fields, demonstrating its significance in understanding chemical phenomena.
Conclusion
This comprehensive guide has delved into the intricacies of determining bond order using molecular orbital theory. We explored the fundamental concepts, including molecular orbital construction, electron configuration, and the interplay between bonding and antibonding orbitals. By analyzing electron density distribution and understanding molecular energy levels, we gained insights into the nature of chemical bonds and their strengths.
Throughout this exploration, several key points emerged. Firstly, molecular orbital theory provides a powerful framework for comprehending bond order by examining the interactions and energies of molecular orbitals. Secondly, the electron density distribution within a molecule offers valuable information about bond strength, as higher electron density indicates stronger bonds. Finally, the limitations of MO theory, such as its assumption of independent electrons, should be acknowledged and addressed through complementary theoretical approaches or experimental validation.
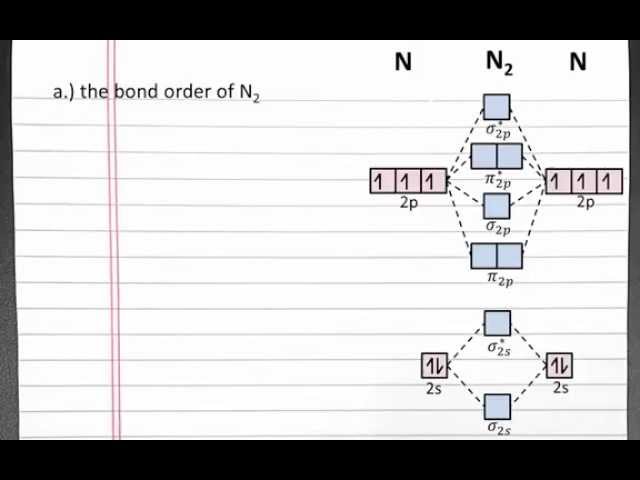